I. INTRODUCTION
In recent times, people are more concerned about their health and appearance. There is therefore a high demand for products formulated with natural and nutraceutical ingredients. Innovative delivery systems are driving the new product development in the field of cosmetics (Patravale & Mandawgade, 2008).1)
Today, consumers are looking for personal care products that supply multiple benefits with minima efforts. A significant number of novel products are based on a new generation of active ingredients. With these emerging actives, come a range of formulation challenges that include stability control and the complications of combining several actives into a single cosmetic product (Fakhravar et al., 2016; Patravale & Mandawgade, 2008).
Liposomes have been extensively studied as drug carriers in the pharmaceutical and medical fields. Research has expanded considerably over the last 30 years, increasing applications area from drug and gene delivery to diagnostics, cosmetics (Fakhravar et al., 2016)2), food and chemical industry. The superiority of liposomes as drug/nutrient carriers has been widely recognized. Several reviews about liposomes as drug delivery systems and specific application via oral, topical, pulmonary, and ophthalmic route have been published.
Liposomes are spherical lipid bilayer surrounding an aqueous core (Edwards, 2016). They are small vesicles composed of phospholipids (Verma et al., 2003). The lipid bilayer of liposomes is composed of double chain, amphiphilic lipids with their nonpolar, hydrophobic tails aligned with each other and their polar, hydrophilic headgroups oriented toward the exterior aqueous solution or directed toward the inner aqueous core (Edwards, 2016). Liposomes can be categorized in different ways based on the diameter and the number of lipid bilayers, liposomes are often classified as small unilamellar vesicles SUVs, 20–100 nm), large unilamellar vesicles (LUVs, 100–1,000 nm), giant unilamellar vesicles (GUVs >1,000 nm, and multilamellar vesicles (MLVs > 500 nm) (Zhang, 2017).
Liposomes can be negatively charged, neutral or positively charged by the addition of other materials (Zhang, 2017) as a certain pH environment (D’Souza, 2017).
Liposomes with a single lipid bilayer are termed unilamellar vesicles and their classification according to size is generally such that small unilamellar vesicles typically have sizes between 20 and 100 nm; those with diameters between 100 nm and 1 μm are generally termed large unilamellar vesicles; and liposomes above 1 μm are termed giant unilamellar vesicle (Edwards, 2016).
Unilamellar liposomes comprise a single bilayer encapsulating an aqueous core. And multilamellar liposomes comprise multiple concentric bilayers with encapsulation possible both within the core and between adjacent bilayers (Edwards, 2016).
The liposomal bilayer is typically composed of a mixture of phospholipids and cholesterol. Phospholipids are amphiphilic molecules consisting of two long fatty acid tails forming a hydrophobic portion, which are linked via esters through glycerol to a phosphate group and hydrophilic headgroup (Edwards, 2016).
Cholesterol imparts reduced permeability to charged and water-soluble molecules by effectively filling in gaps (Edwards, 2016) and enhancing the rigidity of the liposomal bilayer (Lu, 2014).
Lipids have characteristics transition temperature (Tc) which represents the temperature at which they transform from a solid or “gel” phase to liquid or fluid phase. The temperature at which this transition occurs is dependent on the liquid or fluid phase. The temperature at which this transition occurs is dependent on the lipid chain length, degree of unsaturation, presence of chain side groups, as well as the structure of the hydrophilic headgroup. Transition temperature decreases with the degree of unsaturation or side groups in the hydrocarbon chain. Hydration of lipid mixtures above the transition temperature of the composite lipids is necessary to afford a fluid state, which greatly facilitate manipulations required during preparation (Edwards, 2016).
Liposomes are prepared using one of the following methods as reverse phase evaporation, thin film hydration, ethanol/ether injection (Lu, 2014) freeze thaw, dehydration/rehydration (Sulkowski et al., 2005), sonication and detergent dialysis. The choice of method depends on the desired properties of the liposomes and the nature of the material to be encapsulated (Edwards, 2016). Liposomes are used for higher concentration of drugs in deeper layers of skin and a reduction in percutaneous absorption and unwanted side effects (Singh et al., 2014).
These are used as a good delivery vehicle for plant extract. Various extracts can be incorporated into it like turmeric, carrot extract (Singh et al., 2014), papaya extract, aloe-Vera etc. The incorporation of herbal extract into liposome reduces the side effects which are associated with the synthetic drugs (Singh et al., 2014).
The main object was to prepare and characterize liposomal formulations containing Green tea (Camellia sinensis) and Roselle (Hibiscus sabdariffa) calyces extracts as an antioxidant activity indicator and the influence of the extracts on liposome size to be used in cosmetics.
The specific objectives were:
Antioxidants are defined as any substance that delays or inhibits oxidation of a substrate even at low concentrations (Sindhi et al., 2013).
Green tea (Camellia sinensis) has become as one of the most important and commonly consumed herb due to health benefits associated with its high catechin content (Dag & Oztop, 2017; Horie & Kohata, 2000). The major chemical components of the green tea leaf that provides all of these health benefit are polyphenols. Main polyphenols in green tea include gallic acid, quercetin, kaempferol, myricetin and their glycosides (Babu et al., 2008; Dag & Oztop, 2017; <Figure 1>).
Hibiscus sabdariffa L. is a shrub belonging to the family Malvaceae and is popularly known (Bergmeier et al., 2014; Daniele, 2014) as hibiscus or roselle. In botanical terms, it is a thick red plant with fleshly cup-shaped calyxes. The calyxes, rich in phenolic compounds with several physiological activities, also contain large amounts of pectin, anthocyanin, ascorbic acid, malate and protocatechuic acids which may have diuretic and choleretic effects (Bergmeier et al., 2014; Daniele, 2014).
In fact, they decrease blood viscosity, reduce blood pressure and stimulate intestinal peristalsis (Anokwuru et al., 2011; Bergmeier et al., 2014; Daniele, 2014). Roselle extracts have a brilliant red color and are used in the industrial manufacture of jellies, jams, preserves, sauces, beverages, besides being a good source of natural food colorants (ESSELEN; SAMMY, 1975).
Since Roselle extracts contain several polar and acidic compounds, their chemical properties have hampered their use in skin products due to skin irritation and low skin permeation (Hjorth, 1965; Pinsuwan et al., 2010; <Figure 2>).
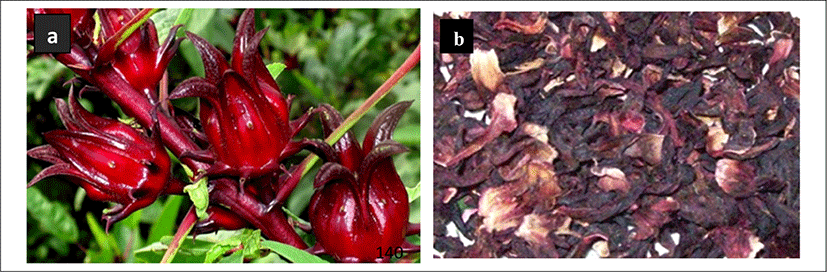
Animal fats and vegetable oils mainly consist of triglycerides that are of glycerin, a trivalent alcohol which is combined with three fatty acids (esterified). When speaking of lipids in today's cosmetic products the term lipid generally is extended to other lipophilic substances like waxes and mineral oils.
A liposome is a tiny bubble (vesicle), made out of the same material as a cell membrane (Choudhury, 2013; Dua et al., 2012). Structurally, liposomes are concentric bleeder vesicles in which an aqueous volume is entirely enclosed by a membranous lipid bilayer. Membranes are usually made of phospholipids, which are molecules that have a hydrophilic head group and a hydrophobic tail group (Dua et al., 2012).
A liposome is a spherical vesicle having at least one lipid bilayer (<Figure 3>). The liposome can be used as a vehicle for administration of nutrients and pharmaceutical drugs. Liposomes can be prepared by disrupting biological membranes (such as by sonication).
Liposomes are used as carriers for numerous molecules in cosmetics, pharmaceuticals, food and farming industries. These industries employ the use of liposome encapsulation to grow delivery systems that can entrap and shield the functionalities of unstable compounds such as antimicrobials, antioxidants, flavors and bioactive elements (Akbarzadeh et al., 2013). Liposomes carry cosmetic agents better into the horny layer and the conditioning effect causes the horny layer to become a depot for these agents (Dragicevic & Maibach, 2016; Lautenschlager). Liposome properties differ considerably with lipid composition, surface charge, size, and the method of preparation (Tejeda-Mansir et al., 2019). Furthermore, the choice of bilayer components determines the ‘rigidity’ or ‘fluidity’ and the charge of the bilayer (Akbarzadeh et al., 2013; Grumezescu, 2018).
The amphiphilic nature of phosphatidylcholine allows them to self-aggregate in an aqueous solution and to form their spherical structures. Liposomes can be unilamellar or multilarmellar and their sizes range from 50 nm to several µm depending on their method of production (New, 1990). They can deliver either hydrophilic (in the aqueous inner core) or lipophilic substances (in the lipid bilayer). Increased rates of skin permeability have been found for various active ingredients, e.g. progesterone and hydrocortisone, when they were applied topically in liposomal form. Also, less frequently side effects were observed when liposomal formulations were employed (Ho et al., 1985; Lasch & Wohlrab, 1986). In contrast to these observations, other investigators could not find improved skin penetration of substances when they were applied in liposomes (Knepp et al., 1990). Also, liposomes themselves did not seem to penetrate through the sc in these early studies (Knepp et al., 1990). The conflicting results may stem from different lipid compositions of the liposomes employed as in addition to size. The lipid composition determines the physical characteristics of the liposomes and, therefore, also the interaction of these carrier systems with the skin (Choi & Maibach, 2005). In the last few years some papers highlighted important factors that influence the penetration of active ingredients encapsulated in liposomes. Liquid-state, flexible liposomes showed greater skin penetration than those in a gel-state, small-sized and unilamellar vesicles seem to result in a higher degree of skin penetration (Blume et al., 2003; Verma et al., 2003). The application form can also influence the penetration kinetics (Cevc & Blume, 1992).
There are four basic stages in all the methods of liposome preparation (Akbarzadeh et al., 2013):
-
Drying down lipids from organic solvent.
-
Dispersing the lipid in aqueous media.
-
Purifying the resultant liposome.
-
Analyzing the final product.
There are two basic techniques for liposome preparation. These are;
Active loading techniques involve three different methods such as
-
Mechanical dispersion method.
-
Solvent dispersion method and.
-
Detergent removal method (removal of non - encapsulated material) (Dua et al., 2012).
The properties of liposomes can be utilized also in the delivery of ingredients in cosmetics. Liposomes offer advantages because lipids are well hydrated and can reduce the dryness of the skin which is a primary cause for ageing. Also, liposomes can supply replenish lipids and importantly linolenic acid to the skin. The first liposomal cosmetic product to appear on the market was the anti-ageing cream “Capture” launched by Christian Dior in 1986 (Muller-Goymann, 2004). Liposomes have been also used in the treatment of hair loss; minoxidil, a vasodilator, is in the active ingredient in products like “Regaine” that claim to prevent or slow hair loss (Lautenschlager, 2006). The skin care preparations with empty or moisture loaded liposome reduce the transdermal water loss and are suitable for the treatment of dry skin. They also enhance the supply of lipids and water to the stratum corneum (Patravale & Mandawgade, 2008). Various liposome formulations were compared in vivo for cosmetics application (Betz et al., 2005) liposome formulations prepared from egg phospholipids exhibited a 1.5-fold increase in skin water content, whereas liposome formulations prepared from soya phospholipids showed no advantage compared to the references. Skin water content was measured daily and the results showed that skin humidity was increased significantly for the formulation containing 20% egg phospholipids during 6 days. Since 1987, several cosmetic products have been commercially available; they range from simple liposome pastes which are used as a replacement for creams, gels and ointments to formulations containing various extracts, moisturizers, antibiotics, etc. Un-rinseable sunscreens, long lasting perfumes, hair conditioners, aftershaves, lipsticks, make-up and similar products are also gaining large fractions of the market (Laouini et al., 2012).
A phospholipid is a lipid that contains a phosphate group and is a major component of cell membranes. A phospholipid consists of a hydrophilic (water-loving) head and hydrophobic (water-fearing) tail. The phospholipid is essentially a triglyceride in which a fatty acid has been replaced by a phosphate group of some sort (<Figure 4>).
The liposomes are primarily composed of phospholipids although other lipids such as galactolipids might also be incorporated into the composition. The phospholipids which is the major components of biological membranes can be divided into two categories (glycerophospholipids and sphingomyelins) according to the alcohols contained in phospholipids.
Glycerophospholipids which are the main phospholipids found in eukaryotic cells contain the glycerol in their backbone. All natural glycerophospholipids are present in α-structure and L-configuration. The head group, the length and the saturation of hydrophobic side chains, the type of bonding between the aliphatic moieties and glycerol backbone, and the number of aliphatic chains determine the chemical structure of glycerophospholipids. According to the head group type, glycerolphospholipids can be grouped as phosphatidylcholine, phosphatidylethanolamine, phosphatidylserine, phosphatidic acid, phosphatidylinositol, phosphatidylglycerol, cardiolipin (Li et al., 2015). In phosphatidylcholine, two acyl hydrocarbon chains are linked to glycerol at the sn-1 and sn-2 position by an ester linkage. The third glycerol carbon linked to a phosphate at the sn-3 position which is then linked to a choline group. The two hydrocarbon chains provide the hydrophobic tail and the hydrophilic phosphocholine provides the polar head group to phosphatidylcholine.
A very important material in the liposomal structure that controls the stiffness is the content of cholesterol. Numerous studies on the use of cholesterol as a stabilizer has been conducted, showing that this steroid can increase the packing of phospholipid molecules improve vesicle resistance to aggregation, change the fluidity of intra-vesicle interactions to make them more rigid and sustain in severe shear stress of the lipid bilayer and reduce drug incorporation efficiency (Briuglia et al., 2015).
The skin is the largest organ of the body, with a total area of about 20 square feet. The skin protects us from microbes and the elements, helps regulate body temperature, and permits the sensations of touch, heat, and cold.
Skin has three layers:
-
The epidermis, the outermost layer of skin, provides a waterproof barrier and creates our skin tone.
-
The dermis, beneath the epidermis, contains tough connective tissue, hair follicles, and sweat glands.
-
The deeper subcutaneous tissue (hypodermis) is made of fat and connective tissue.
The skin’s color is created by special cells called melanocytes, which produce the pigment melanin. Melanocytes are located in the epidermis.
The liposome stability is a major concern needed to be taken into consideration for the liposome formation, storage and delivery. Thus, the physical and chemical stability of the liposomes should be monitored by characterization of the liposome over a certain time period. Generally, the physical stability refers to the preservation of liposome structure characteristics while the chemical stability is related to the change in molecular structure of liposomes (Chrai et al., 2002). The zeta potential, average particle size, polydispersity index, encapsulation efficiency, lamellarity determination, phase and quantification of the residual solvent are the most common parameters used in the characterization of liposomes (Laouini et al., 2012). A detailed description of the methods used in the present study are given in the following sections.
Due to the fact that most particle size determination methods have difficulties to differentiate individual and aggregated liposome, microscopic observation of the liposome is required in order to confirm particle size measurement and to gain valuable information about the structure of the liposomes. Although light microscope provides several advantages of obtaining vesicle images in a short time using the standard laboratory equipment, information about the vesicle morphology, the sample heterogeneity regarding the shape and size, and differentiation of single and aggregated liposome it is inadequate to gain comprehensive information about the lipid bilayer of liposomes which is offered by other microscope techniques (Bibi et al., 2011).
Electron microscope offers greater magnification which allows to obtain information about lipid bilayer characteristics and the visualization of structure of much smaller unilamellar vesicles which cannot be visualized by light microscope. More specifically, while light microscopes have a resolution of 200 nm, electron microscopes offer a resolution about 0.2 nm. The common electron microscopes used in the characterization of liposome are transmission electron microscope which gives the information about internal structure of particles and scanning electron microscope indicating surface morphology of the particles. In the most basic terms, electron microscope working principle is based on the exposure of the sample to an electron beam which is focused by various lenses. Some electron then collide and displace electrons around the nuclei of atoms in sample while the rest of electron change their path only. Eventually, a projected image is created by focusing and magnifying electrons using a system of magnetic lenses (Bibi et al., 2011).
II. MATERIALS AND METHODS
The dried calyces of Hibiscus sabdariffa (Roselle) and Camellia sinensis (Green tea) were obtained from Madina market, La Nkwatanan district in the Greater Accra region in Ghana and SEO LIM Scientific systems in Korea respectively as shown in <Table 1>.
Common name | Scientific name | Quantity | Producer/company |
---|---|---|---|
Roselle | Hibiscus sabdariffa | 500 g | Purchased in Madina market from Ghana |
Green tea | Camellia sinensis | 500 g | Super Food Ltd. |
Before extraction process, both samples were divided into two halves and ground using a household blender (<Figure 5>).
Phosphatidylcholine (Soybean Lecithin) and cholesterol were obtained from SEO LIM Scientific systems <Table 2>, <Figure 5>.
Name | Abbreviation | Charge | Producer/company |
---|---|---|---|
Cholesterol | CHO | No charge | Aldrich |
Phosphatidylcholine (soybean lecithin) | SL | +/- | Aldrich |
All chemicals and reagents were high purity grade (<Table 3>).
Liposomes were prepared by Reverse phase evaporation method. Each material was accurately weighed and placed into two separate vessels, one for the aqueous phase and one for the organic phase (chloroform). The two phases were mixed together to form water in oil (w/o) emulsion (Reverse micelles). The organic solvent was evaporated to form a gel, the reverse micelles then collapses to form a suspension. The suspension is re-dissolved a second organic solvent (methanol) and the solvent is evaporated under reduced pressure to form liposome. The volume is finally readjusted with water.
III. RESULTS AND DISCUSSIONS
<Figure 6> shows the physical appearances of Roselle calyces and grinded calyces extracted with different solvents. They are labelled as follows: (a) ethanolic extraction of whole Roselle calyces (GHFE), (b) ethanolic extraction of grinded Roselle calyces (GHPE), (c) water extraction of grinded Roselle calyces (GHPW), (d) water extraction of whole Roselle calyces (GHFW), (e) water, ethyl alcohol and chloroform extraction of whole Roselle calyces (GHFWC) and water, ethyl alcohol and chloroform extraction of whole Roselle calyces (GHPWC). All extracts show bright red in appearance except for GHPW & GHFW which shows dark red in color. The % average yield of the extracts are as follows: GHFE (49.97 ± 1.4), GHPE (52.88 ± 8), GHPW (51.49 ± 1.5), GHFW (45.26 ± 13.8), GHFWC (52.42 ± 4), GHPWC (56.06 ± 1.9).
<Figure 7> shows a bar graph of percentage average yield of Roselle extract, GHPWC has the highest yield with 56.06 ± 1.9% whiles the water extraction of the whole calyces GHFW has the lowest yield with 45.26 ± 13.8%.
<Figure 8> shows the physical appearances of whole green tea leaves and grinded green tea leaves extracted with different solvents. They are labelled as follows: (a) ethanolic extract from whole green tea leaves (GTLE), (b) ethanolic extract from grinded green tea leaves (GTPE), (c) water extract from grinded green tea leaves (GTPW), (d) water extract from whole green tea leaves (GTLW), (e) water, ethyl alcohol and chloroform extract from whole green tea leaves (GTLWC) and (f) water, ethyl alcohol and chloroform extract from grinded green tea leaves (GTPWC).
The GTLE & GTPE are greenish with % average yield of 33.66 ± 0.23 & 34.86 ± 3.17. The GTPW & GTLW are light green in appearance with % average yield of 27.83 ± 1.38 & 30.35 ± 0.9.
GTLWC & GTPWC extracts shows brownish green in appearance with % average yield of 19.22 ± 1.20 & 19.42 ± 2.28 respectively.
<Figure 9> shows a bar graph of percentage average yield of Green tea, GTPE has the highest yield with 34.86 ± 3.17% whiles GTLWC has the lowest yield with 19.22 ± 1.19%.
<Figure 10> shows a bar graph of TPC of Roselle extracts from different solvents. TPC of the various Roselle extracts are as follows: GHPW (54.57 ± 0.11 mgGAE/g), GHFW (60.85 ± 0.21 mgGAE/g), GHPE (60.38 ± 0.21 mgGAE/g), GHFE (53.78 ± 0.17 mgGAE/g), GHFWC (45.49 ± 0.34 mgGAE/g), GHPWC (34.89 ± 0.34 mgGAE/g).
GHFW has the highest bar with TPC of 60.85 ± 0.21 whiles GHPWC has the lowest bar with 34.89 ± 0.34 mgGAE/g.
<Figure 11> shows a bar graph of TPC of Green tea extracts from different solvents. TPC of the various Green tea extracts are as follows: GTPWC (179.63 ± 0.81 mgGAE/g), GTLWC (302.49 ± 0.17 mgGAE/g), GTLW (332.73 ± 0.13 mgGAE/g), GTPW (280.55 ± 0.15 mgGAE/g), GTPE (248.58 ± 0.15 mgGAE/g), GTLE (237.73 ± 1.04 mgGAE/g).
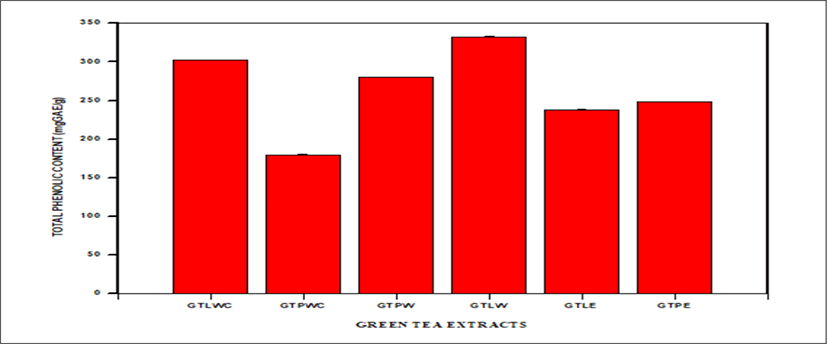
GTLW has the highest bar with TPC of 332.73 ± 0.13 mgGAE/g whiles GTPWC has the lowest bar with TPC of 179.63 ± 0.81mgGAE/g.
All extracts of Roselle show bright red in appearance except for GHPW & GHFW which shows dark red in color. GHPWC has the most abundant yield with 56.06 ±1.9% whiles GHFW has the lowest yield with 45.26 ± 13.8%.
The GTLE & GTPE are greenish with % average yield of 33.66 ± 0.23 & 34.86 ± 3.17. The GTPW & GTLW are light green in appearance with % average yield of 27.83 ± 1.38 & 30.35 ± 0.9. GTLWC & GTPWC extracts shows brownish green in appearance with % average yield of 19.22 ± 1.20 & 19.42 ± 2.28 respectively.
GHFW has the highest bar with TPC of 60.85 ± 0.21 whiles GHPWC has the lowest bar with 34.89 ± 0.34 mgGAE/g.
GTLW has the highest bar with TPC of 332.73 ± 0.13 mgGAE/g whiles GTPWC has the lowest bar with TPC of 179.63 ± 0.81 mgGAE/g.
TPCs of Roselle extracts from different solvents were found to be GHPW; 54.58 ± 0.01 mgGAE/g, GHFW; 60.85 ± 0.04 mgGAE/g, GHPWC; 34.89 ± 0.05 mgGAE/g, GHFWC; 45.50 ± 0.06 mgGAE/g, GHFE; 53.78 ± 0.17 mgGAE/g, GHPE; 60.39 ± 0.08 mgGAE/g. These values are higher than previously reported results of total polyphenolic contents of Hibiscus sabdariffa calyx by (Anokwuru et al., 2011). Results evidenced that extract yielded 29., 27.6, 19.3, and 27.6 mg GAE g-1 respectively with methanol, ethanol, acetone and water as solvents. (Dalar et al., 2012) reported maximum levels of total phenols of 17.4 ± 0.3 mg GAE g–1 of dry weight of the lyophilized powder and 35.3 ± 2.8 mg GAE g-1 of dry weight of the lyophilized powder in acidified methanolic extracts of Malva neglecta and Plantago lanceolata, respectively. TPCs were however similsr to the results obtained by (Medina et al., 2011) studies on the effect and activity of araca extracts as antioxidant, antimicrobial and anti-proliferative features on human cancer cells. (Wootton-Beard et al., 2011) investigated total polyphenol content of 23 commercially available vegetable juices before and after in vitro digestion.
In general, the results obtained in current assays indicated that the extraction of total polyphenols contents was maximized and the compounds were superior to other plants reported in the literature. Bars represent the means of experiments carried out in triplicates.
Statistical Analysis. Data were expressed as the means (standard error of the means of the experiments carried out in triplicates. A one-way ANOVA single factor was used to evaluate the significance of results. A probability (p) value <0.05 was considered significant.
<Figure 12> shows the physical appearances of empty liposome formulations as follows:
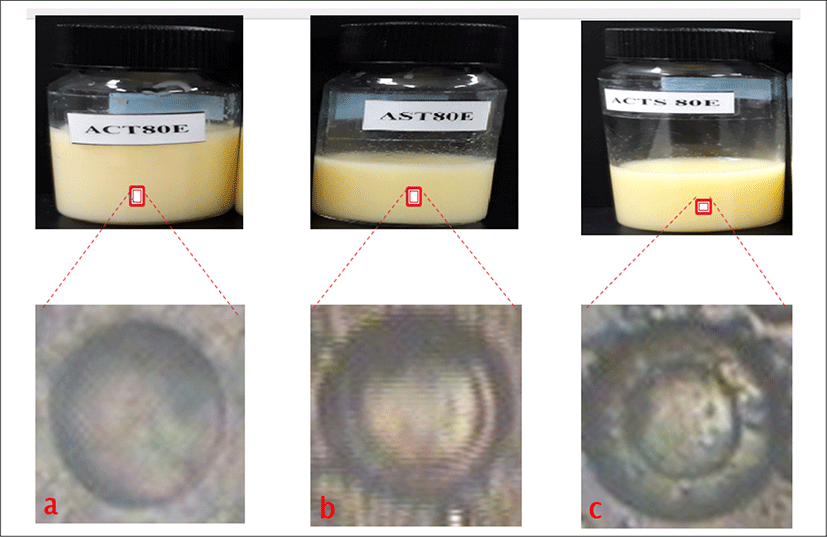
-
is empty liposome containing only cholesterol with lipid concentrations as 80 μmole/mL (ACT80E).
-
is empty liposome with lipid concentrations as 80 μmole/mL (AST80E).
-
is empty liposome containing cholesterol with lipid concentrations as 80 μmole/mL (ACTS80E). All formulations gave milky to yellowish colloidal appearance depending on the amount of total lipid content in formulations. The liposome with lowest total lipid content exhibited the milky colloidal appearances. Also, the formulations with the highest total lipid content showed the yellowish colloidal appearances. No precipitation and phase separation were observed in day 1.
<Figure 12> shows the morphology of empty liposome formulation using phase contrast microscope. a is ACT80E has a thinner shell/wall with large aqueous core, b is AST80E which indicates a large aqueous core with slightly thicker and larger wall, c is ACTS80E and the image forms has thicker and larger wall/shell with slightly bigger aqueous core.
<Figure 13> shows the physical appearances of all Green tea loaded liposome formulations as follows:
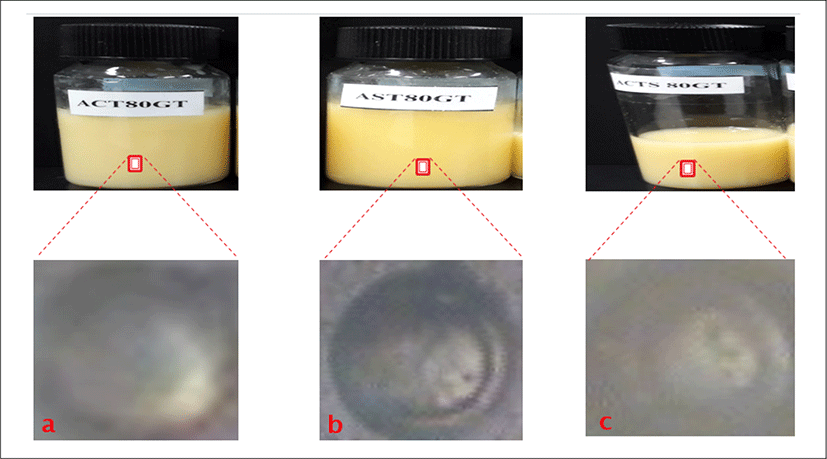
a is empty liposomes containing cholesterol and green tea extract with lipid concentrations of 80 μmole/mL (ACT80GT). b is empty liposomes containing Green tea extract with lipid concentrations of 80 μmole/mL (AST80GT). c is empty liposomes containing cholesterol and Green tea with lipid concentration of 80 μmole/mL (ACTS80GT).
The physical appearances of all the Green tea loaded liposomes formulations gave milky, slightly yellowish to yellowish colloidal appearance depending on the amount of total lipid content in formulations. The Green tea loaded liposome with cholesterol and the lowest total lipid content exhibited the milky colloidal appearances. The formulation without cholesterol but with lowest lipid content shows slightly yellowish colloidal appearances. Also, the formulations with the highest total lipid content showed the yellowish colloidal appearances as shown in the formulations ACT80GT, AST80GT, and ACTS80GT. No precipitation and phase separation were observed in day 1.
<Figure 12> shows the morphology of Green tea loaded liposome formulation with concentration of 80 μmol/mL using phase contrast microscope. a is (ACT80GT) and has thinner shell/wall with large aqueous core, b is (AST80GT), which indicates a large aqueous core with slightly thicker and larger wall, c is (ACTS80GT) and the image forms has thicker and larger wall/shell with slightly bigger aqueous core.
<Figure 14> shows the physical appearances of Roselle loaded liposome formulations as follows: a is empty liposomes containing cholesterol and Roselle extract with lipid concentration of 80 μmol/mL (ACT80HB). b is empty liposomes containing Roselle extract with lipid concentration of 80 μmol/mL (AST80HB). c is empty liposomes containing cholesterol and Roselle extract with different lipid concentration of 80 μmol/mL (ACTS80HB).
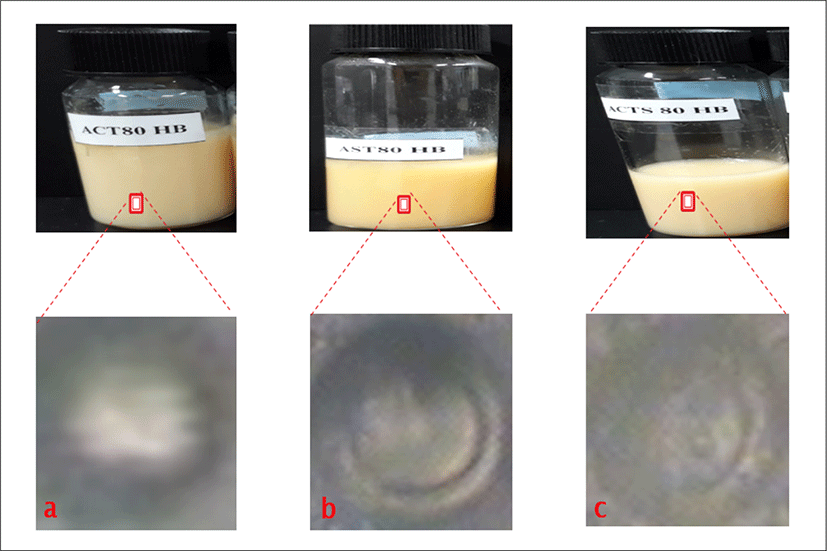
The physical appearances of all Roselle liposome formulations gave pinkish to yellowish colloidal appearance depending on the amount of total lipid content in formulations. The Liposome with cholesterol and the lowest total lipid content exhibited pinkish colloidal appearances as shown in the formulations. The rest of the formulations showed slightly yellowish or yellowish colloidal appearances as in AST80HB and ACTS80HB. No precipitation and phase separation were observed in day 1.
<Figure 14> shows the morphology of Roselle loaded liposome formulation with concentration of 80 μmol/mL using phase contrast microscope. a is (ACT80HB) and has thinner shell/wall with large aqueous core, b is (AST80HB), and c (ACTS80HB) indicate large aqueous core with slightly thicker and larger wall.
<Figure 15> shows the physical appearances of liposome formulations as follows: a is empty liposomes containing only cholesterol with lipid concentration of 80 μmol/mL (ACT80E). b is empty liposomes containing cholesterol and Green tea with lipid concentration of 80 μmol/mL (ACT8GT). c is empty liposomes containing cholesterol and Roselle extract with lipid concentration of 80 μmol/mL (ACT80HB). <Figure 15> shows the morphology of different liposome formulations with concentration of 80 μmol/mL using phase contrast microscope. All the three a (ACT80E), b(ACT80GT), and c (ACT80HB) have thinner shell/wall with large aqueous core.
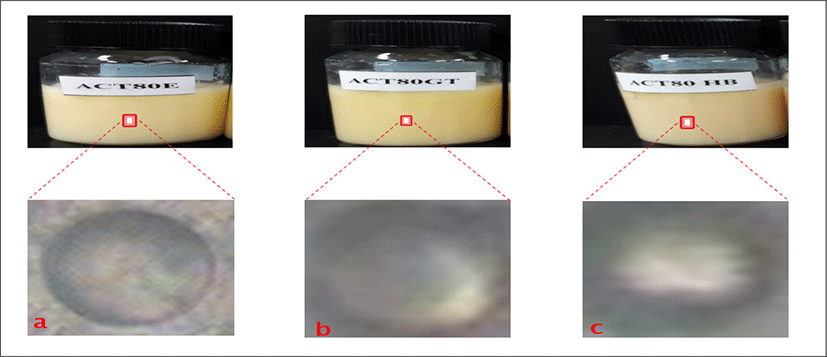
<Figure 16> shows the morphology of different liposome formulations with concentration of 80 μmol/mL using phase contrast microscope. All the three a (ACTS80E), b(ACTS80GT), and c (ACTS80HB) have large and thicker shell/wall with slightly larger aqueous core.
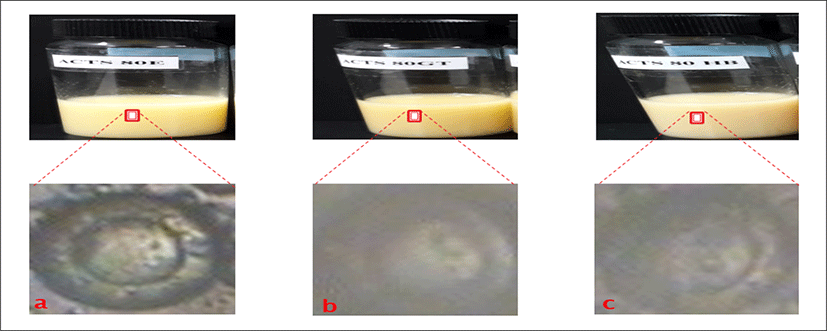
Size and size distribution (polydispersity) of the formulated liposomes are of particular importance in their characterization. Maintaining a constant size and/or size distribution for a prolonged period of time is an indication of liposome stability.
In this experiment microscopic methods and dynamic light scattering were used for establishing the morphology, size and stability of liposome.
The changes in the morphology of liposomes obtained by natural swelling in the presence of cholesterol. The mean particle size of green tea and Roselle extract loaded liposomes prepared by reverse phase evaporation method was measured to investigate the stability of the systems. Previous studies indicate the mean particle size was highly dependent on the composition of liposomes. In the study carried by (Gibis et al., 2012) it was concluded the size of the liposomes depended on the material that was encapsulated into liposomes.
The higher mean particle size of the extract loaded liposomes result might be explained by the fact that phenolic compounds might be incorporated into a lipid bilayer and/or may be absorbed onto the surface of liposomes as well as incorporated into the interior region of the liposomes which could be due to hydrogen bonding between polar head groups and the phenolic compounds in the extract, hydrophobic interactions between the fatty acid tail so of the polar lipid and the more hydrophobic moieties of the phenolic compounds, or thermodynamic driving forces such as the liposomes attaining a more optimal configuration (Gibis et al., 2012).
IV. CONCLUSION
In conclusion, Green tea and Roselle extracts are natural antioxidants rich in polyphenols. The role of polyphenols from Green tea and Roselle extracts have great deal of potential as part of a growing natural antiaging and whitening skin care market with all-natural ingredients however, it is important to standardize extraction methods as well as their assay for their activity.
In the scope of this study, the Roselle and the green tea extract were encapsulated into liposomes by dispersing soy lecithin in distilled water by Reverse phase evaporation method in order to enhance the stability of polyphenols and protect their functional properties.
It was found that the Liposomes produced with only cholesterol gave a larger aqueous core with thinner wall/shell and most stable formulations. The formulations with Cholesterol gave the smallest aqueous core but thicker wall/shell.
The mean particle size of the extract loaded liposomes were high in almost all cases than the empty liposomes.
The higher mean particle size of the extract loaded liposomes might be explained by the fact that phenolic compound might be incorporated into lipid bilayer and/or may be absorbed onto the surface of liposome due to hydrogen bonding between polar head groups and the phenolic compounds in the extract (Gibis et al., 2012).